Tracing the Evolution of Our Universe through Blackbody Photon Dynamics
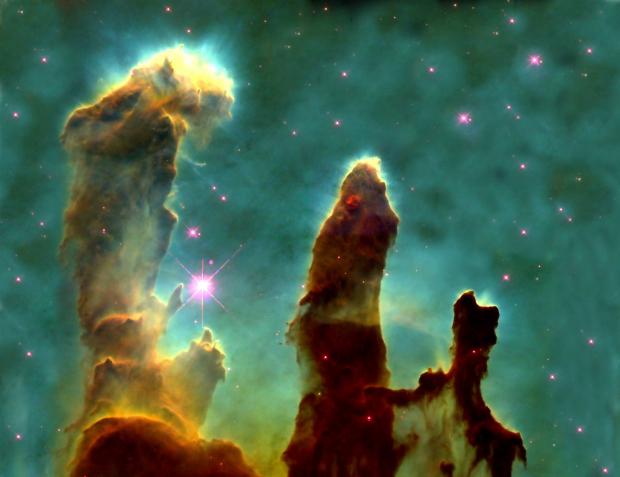
Abstract
How was our universe actually created? Its history can be understood by examining the elementary particles and photons that were present at the start of its creation. Studying these moments reveals that the universe was at a level of non-uniformity, or anisotropy, and today it has an inhomogeneous structure. As a result of changing energy densities, photons scattered, a process that is not yet fully understood. This paper examines an outline of the universe’s evolution and then analyzes the dynamics of the particles at that time to explore several causes of anisotropy. Compton scattering, one method of photon scattering, helps explain the current state of the universe by looking at the relationships between subatomic particles. Finally, by studying the effects of these tiny objects, the evolution of the universe can be tracked from the beginning until modern day.
Just under 13.8 billion years ago, our universe was an infinitesimally tiny dot. A little less than 400,000 years after that, however, it had become a hot, dense, highly ionized plasma with a temperature of about 5000 degrees Fahrenheit and a density about 109 times the current value (1). Then, something fascinating happened. The plasma underwent a rapid process called recombination, with protons attaching to electrons to form hydrogen, emitting photons with each reaction, and providing the footprints of today’s universe (2). One of the most interesting aspects of the study of these early moments has to do with the non-uniformity of the universe. This non-uniformity, or anisotropy, as it is technically called, is reflected in the inhomogeneous structure of the current universe. During the early moments of recombination, energy density fluctuations triggered the scattering of photons. Yet, what we do not fully comprehend are the dynamics of how this scattering occurred. As the universe expanded, this same inhomogeneity magnified, meaning that by studying the original scattering in the photosphere during the moments of recombination, we can largely understand the current structure of the universe (2). The modern universe and the microwave background remnant radiation that we observe are thus just “image[s] of the surface of [the] last scattering” which occurred about 378,000 years following the Big Bang (1). Accordingly, in this paper, an overview of the evolution of the universe will first be given. Then, the dynamics of the universe’s elementary particles and photons at the time of recombination will be analyzed and some of the possible causes of anisotropy will be examined. After that, there will be a discussion of the possibilities of how one type of scattering known as Compton scattering could be used to help describe the non-uniform structure of the present-day cosmos to a greater extent by taking into account interactions between massive subatomic particles like protons, electrons, and massless photons. By understanding this aspect of inhomogeneity and scattering, we will be able to give a detailed and accurate account of how the cosmos has evolved. Thus, remarkably, by studying the dynamics of some of the most microscopic objects we know of, we will be able to trace the history of the universe itself, from the beginning of time up until today.
Before embarking on this journey, it is first important to understand a little more about cosmic microwave background radiation, which provides the most important information to modern researchers about the history of our universe. The cosmic microwave background radiation, or CMB, is a remnant radiation from the early universe with a current density of about 400 photons per cubic centimeter and a temperature of approximately 2.7 K (3). It is the driving force behind modern cosmological study as it provides clues about how the universe began and why and how it has evolved as it has. In the early 1990s, NASA’s Cosmic Background Explorer mission, or COBE, analyzed this radiation, and found that it likely came from an ionized plasma consisting primarily of subatomic particles and light energy that existed in that state until about 378,000 years after the beginning of the universe (4). COBE’s initial measurement had a low deviation from the spectrum that a uniform universe would have produced when the radiation was emitted. However, higher precision results on the mission and in future projects have shown variations from this ideal pattern. These variations are considered the seeds of the non-uniformity, or anisotropy, seen in today’s universe (3). Considering that COBE observations gave us a wealth of information about the Big Bang and the very early universe, studying the nature of anisotropies has become arguably the most important topic of research in cosmology today. However, before delving into discussion about the proposed theories behind the various causes of anisotropy, we will look first at an overview of the evolution of the universe.
According to accepted scientific theory, the universe began in a very hot, very dense stage following the outward explosion at the beginning of time called the Big Bang; the expansion that began then still continues today at an accelerating pace (5). Early in the universe’s history, the temperature was too high for atoms to stay in a bonded state. As the universe expanded and cooled, however, light elements such as deuterium, a simple form of hydrogen, slowly started to form. With time, these elements then fused into larger, heavier elements. Along with matter which consists of these elements, the present universe is pervaded by dark energy, a form of energy that has not yet been directly detected but scientists have inferred that it exists due to its effects on galaxy structures. Dark matter, a type of matter which is thought to not reflect or emit any radiation, is also theorized to exist based on the gravitational forces experienced by visible bodies in deep space. With regards to the structure of the universe itself, anisotropies exist on various scales. As previously discussed, these anisotropies are also manifested in the CMB radiation that permeates the universe (6).
Given this big picture, we can now look more closely at some of the details behind how these anisotropies may have come to exist. Firstly, gravitational redshifts caused anisotropies. This gravitational redshift is a consequence of the Einstein’s Theory of General Relativity, where the moving of electromagnetic radiation energy from an area of high gravity force to an area of low gravity force causes an increase in wavelength, also called redshift. The overall result is called the Sachs-Wolfe effect (5). The increase in wavelength due to this effect meant that some areas of the radiation varied from their original spectral forms, creating non-uniformity. Secondly, a phenomenon called radiation pressure arose from highly energetic photons acted on by gravity-produced oscillations very similar to the oscillations observed in acoustics. These oscillations took place before the recombination of protons and electrons into neutral atoms. At the moment of recombination of a given atom, the phase of the radiation pressure oscillation was set in place. Taken together, the variations in the phases of these oscillations created its own non-uniformity (5, 8). Thirdly, since recombination was not instantaneous, some of the recombining particles were deflected by atoms that had already combined, lowering, or dampening, the momentum of some of the particles. This effect caused extra anisotropy, scientifically referred to as the “damping tail” (5). Fourthly, a so-called “large” anisotropy component exists in the observed cosmic microwave background and comes about due to the fact that the Earth and its satellites, where CMB is measured from, are moving relative to the radiation (3). Thus, as is apparent, there are numerous reasons behind why anisotropy exists. However, despite accounting for all of the causes that have been given, scientists have still not been able to fully explain the non-uniformity of the CMB and our universe. To try to solve this problem, it is essential to understand another possible cause of anisotropy, Compton scattering.
Compton scattering was discovered by Arthur H. Compton more than a century ago. Compton observed how x-rays scatter after hitting electrons in a carbon target. Through experimentation, he found that the scattered x-rays that left the target had a longer wavelength than those that had not yet hit the target. To try to make sense of this phenomenon, Compton used the assumptions of the dual particle and wave nature of light, based on the idea of the photon travelling in wave patterns. He realized that when the photon hits an electron, it must transfer some of its energy to the electron. However, since photons do not have mass, he concluded, based on empirical results, that the loss of energy is manifested as an increase in the wavelength of the photon. This conclusion agreed with the theories of quantum mechanics which state that the quantum energy of a photon is inversely proportional to its wavelength. Given this understanding of the energy dynamics in massless photons, Compton could apply the laws of conservation of energy and conservation of momentum between the photon and the electron to arrive at his final equations for Compton scattering (14). In the context of CMB, the results of Compton scattering have been more generally termed the Sunyaev-Zel’dovich (SZ) effect (5). However, the study of the SZ effect is quite complex and thus, before delving into the interactions of particles due to the SZ effect, we should first try to understand how the universe would have looked at the time of recombination that we are interested in. Once this is understood, we can then look more closely at how exactly the universe would have been altered at that time so as to cause the non-uniformity we observe today.
During the time of recombination, the universe was close to being a homogeneous plasma in thermal equilibrium (10). The behavior of such a universe was theorized to be like that of a special type of object called a blackbody. Any variations from the ideal blackbody properties are the most critical clues about the nature of our universe’s non-uniformities. Thus, it is important to examine them in some detail first. A blackbody is a unique object that absorbs the energy of all incoming electromagnetic radiation into its subatomic particles. Later, due to internal energy processes, these subatomic particles re-emit that energy as electromagnetic radiation. This process is different from reflection, where electromagnetic radiation merely bounces off objects without transferring all its energy to them. All blackbodies display the following properties: firstly, they absorb all energy that is incident on them from any direction and at any wavelength; moreover, for any given wavelength and temperature, they radiate more energy than any other type of body at the same temperature; lastly, they emit energy equally in all directions; that is, they are “diffuse” emitters (12). Using the quantum mechanical principles which govern the way in which discrete energy levels inside atoms work, Max Planck discovered that all blackbodies emit radiation in a specific pattern of spectral frequencies. This special form can be can be described precisely by Planck’s radiation law, which shows how the intensity of radiation at a certain wavelength emitted by a blackbody is only dependent on the temperature, and thus, the total amount of energy inside the blackbody (13). Any deviations from an ideal blackbody spectrum would also show deviations from adherence to Planck’s radiation law. Thus, if the blackbody model of the early universe were correct, the radiation emitted by it during recombination, called cosmic microwave background radiation or CMB, should follow this blackbody spectral form described by Planck. As previously noted, in the 1990s, NASA’s COBE mission was able to precisely measure the remnant of these early photon emissions, which, remarkably, displayed an almost exact blackbody spectrum pattern while pervading the Universe in the form of CMB (11).However, COBE also was able to measure minute CMB temperature fluctuations which are a reflection of the non-uniformity of the Universe. Given the information now known about blackbodies, we analyze the exact interactions of Compton scattering in the context of the early blackbody universe. This should give a more complete understanding of how the SZ effect contributed to the cosmos’s anisotropy.
At the time when CMB began, the Universe was primarily made up of protons, electrons, and photons, along with some other non-interacting particles such as dark matter. At this point, most of the electrons and protons were in a ‘free’ state, meaning that they were not bonded as part of any atoms. Thus, they would absorb any energy that hit them. As a result, most of the electromagnetic radiation at the time would have been fully absorbed and re-emitted by the subatomic particles. Since this complete absorption and re-emission by electrons and protons is the defining characteristic of blackbodies, most of this radiation took the form of a blackbody spectrum (15). However, there was still more to this dynamic. Simultaneously, Compton scattering would have been occurring. To understand how this would have taken place, let us first look more closely at the process of recombination which was acting during the era of the universe that we are analyzing. Recombination involved protons and electrons coming together to form hydrogen atoms. At this point in the history of the universe, the temperature was finally cool enough that this bonding could happen (16). When a proton and electron bonded to form hydrogen, energy was released in the form of a photon. The photon would travel through the recombining universe and usually hit the vast array of protons and electrons that were in an un-bonded ‘free’ state. These free subatomic particles would absorb all the energy of these photons and re-emit that energy as radiation, forming the essence of the blackbody-form microwave background radiation that is observed today (15).
Figure 1. Distortion caused by Compton Scattering
Yet, while the free-state protons and electrons of the early universe completely absorbed and re-emitted the energy of the photons produced during recombination, there were still a number of bonded atoms in existence. Whenever photons struck these bonded atoms, their energy would not be completely absorbed and thus would not later be completely re-emitted. Instead, the atoms would absorb a little of the energy and reflect the photons. In this process, the photons loss of energy would be manifested as an increase in its wavelength. The net result of this Compton scattering was that the radiation spectrum would not follow the perfect blackbody form that it would have if only “free,” un-bonded subatomic particles existed. The distortion caused by this Compton scattering to the blackbody photons and spectrum can be seen in Figure 1. Thus, overall, the early universe did not act like a perfect blackbody, and this had some important implications. Most significantly, because of these imperfections, the early universe did not distribute energy equally in all directions, as opposed to a perfect blackbody, which, due to its ‘diffuse’ emission properties would radiate equally in all directions, as described earlier. The early inhomogeneity originating from the process of Compton scattering is thus likely to be a cause for the inhomogeneity that has ballooned into the non-uniform structure of today’s universe.
Yet, there is a little more along the lines of Compton scattering that needs to be taken into account. Another type of Compton scattering, called double-photon Compton scattering, must be explored. This is because, in the low frequency range of radiation, relativistic effects need to be taken into account, in addition to the quantum mechanical effects of energy transfer between photons, protons, and electrons described by normal Compton scattering. As Chluba suggests, by examining this effect as well, “we [can] obtain the necessary tools” to get a more complete picture of the various distortions of the cosmic microwave background in the early universe (17). Due to the relativistic effects of mass-energy conversion, it has been shown that the double-photon Compton (DC) scattering emission power increases at a significantly slower rate with temperature. By correcting for these DC scattering effects in the equations that thus far only describe distortions due to normal Compton scattering, the distortion caused by the relativistic effects can also be accounted for (10, 17).
Figure 2. Cosmic background spectrum at the north galactic pole
Thus, this study has provided an analysis of how the processes of Compton scattering and double-photon Compton scattering would have affected the dynamics of the recombination universe, causing distortions which can be seen 13.8 billion years later in the form of a non-uniform cosmos. Studying these dynamics closely gives us a much better idea of why the universe is structured as it is today and allows us to better hypothesize what we should be looking for when studying deep space and galactic structures.
This analysis is especially significant in the larger scheme of research in astrophysics because the blackbody model of the universe is so widely accepted. As seen in Figure 2, the CMB radiation was within 1% of the theorized blackbody spectrum in COBE’s results, and thus, almost all research about anisotropy today assumes this blackbody baseline. Given that this study also uses this blackbody framework, the analysis about Compton scattering is very likely to make an impact. Still, however, the manifested effects of these CMB distortions are small and NASA’s COBE data were not quite sensitive enough to fully detect them. Based on the lower level of constraints of the new PIXIE mission, the possibility of reconciling these synthesized theories with experimental data seems likely (11). If not PIXIE, then the ongoing Planck mission might also be sufficient to serve this goal. Even if this data is not fully reconciled with theory soon, research into CMB should help boost studies to understand other aspects of the evolution of our universe, such as inflation, which is the ultra-rapid expansion of the universe that occurred in the instants after the Big Bang. For two reasons, the potential for groundbreaking discoveries in cosmology is ripe. Firstly, new technology has been developed for measuring the “large” component of anisotropy discussed earlier and has also been found to be useful in other aspects of cosmological study (18). Secondly, the very recent finding of gravitational waves, an elusive remnant of the early universe predicted by Einstein, provides a starting point for several new theories that could be tested using existing technology (19). Nevertheless, until then, based on the analysis presented in this paper, we can at the least get a synthesized idea of the Compton scattering theories behind understanding how the universe has evolved from a tiny dot of energy 13.8 billion years ago into the vast expanse of stars, galaxies, and novae that we observe today.
Sources
1. Kosowsky A. 2006. The cosmic microwave background. Italian Society of Gravitational Physics Summer School; 2000 May; Como, Italy. Piscataway: Rutgers University Press. 1-52.
2. White M, Scott D, Silk J. Anisotropies in the cosmic microwave background. Annual Review of Astronomy and Astrophysics. 1994;32:319-370.
3. de Bernardis, P. The cosmic microwave background: a window on the early universe. Nuclear Physics B – Proceedings and Supplements. 2013;243:33-43.
4. Chluba, J, Jeong D. Teasing bits of information out of the CMB energy spectrum. Monthly Notices of the Royal Astronomical Society. 2014;438(3):2065-2082.
5. Scott D, Smoot G. Cosmic microwave background. Astrophysics, Comsology, and Extragalactic Physics. 2010;1-8.
6. Vogeley M, Ratra B. The beginning and evolution of the universe. Publications of the Astronomical Society of the Pacific. 2008;120(865):235-265.
7. Sachs R, Wolfe A. Perturbations of a cosmological model and angular variations of the microwave background. 1967;147:73-90.
8. Wayne Hu: CMB Introduction [Internet]. Chicago (IL): University of Chicago (US); [updated 2014]; [about 4 p.]. Available from: http://background.uchicago.edu/~whu/physics/acoustic.html
9. Rephaeli, Y. Comptonization of the cosmic microwave background: the Sunyaev-Zeldovich effect. 1995;33(1):541-579.
10. Opher M, Opher R. Was the electromagnetic spectrum a blackbody spectrum in the early universe. Physical Review Letters. 1997:79(1):2628-2631.
11. Smoot G, Scott D. Cosmic background radiation. European Physical Journal. 2000;15(1):145-149.
12. Zel’dovich Y, Kurt V, Syunyaev R. Recombination of hydrogen in the hot model of the universe. Soviet Physics JETP. 1969;28(1):146-150.
13. Planck M. The theory of heat radiation. Worcester: Forgotten Books; 2012.
14. Massoud M. Engineering and thermofluids: thermodynamics, fluid mechanics, and heat transfer. Berlin: Springer; 2005.
15. Yuki D. Takahashi: Big bang – how did the universe begin [Internet]. Thomson scattering [Internet]. Pasadena (CA): California Institute of Technology (US); [update 2000]; [about 7 p.]. Available from:http://www.ugcs.caltech.edu/~yukimoon/BigBang/BigBang.htm.
16. Khatri R, Sunyaev R, Chluba J. Mixing of blackbodies: entropy production and dissipation of sound waves in the early universe. Astronomy & Astrophysics. 2012;543:136-151.
17. Chluba J. Spectral distortions of the cosmic microwave background [dissertation]. Munich (Germany): Ludwig Maximilian University of Munich; 2005.
18. Bock J, Church S, Devlin M, Hinshaw G, Lange A, Lee A, Page L, Partridge B, Ruhl J, Tegmark M. Task force on cosmic microwave background research. 2005;1-87.
19. Elizabeth Landau: Big Bang breakthrough announced; gravitational waves detected [Internet]. Atlanta (GA): CNN (US); [updated March 18, 2014]; [about 4 p.]. Available from:http://www.cnn.com/2014/03/17/tech/innovation/big-bang-gravitational-waves/